JACK STEINBERGER PHYSICIST NOBEL PRIZE WINNER HADRON NEUTRINO MANUSCRIPT QUARKS For Sale
When you click on links to various merchants on this site and make a purchase, this can result in this site earning a commission. Affiliate programs and affiliations include, but are not limited to, the eBay Partner Network.
JACK STEINBERGER PHYSICIST NOBEL PRIZE WINNER HADRON NEUTRINO MANUSCRIPT QUARKS:
$16365.78
Handwritten manuscript signed three pages8.25x11.75 inches circa1968 complex scientific manuscript headed "Probability that an electron, energy Ee will be counted as a hadron", written and signed in blue ink "J. S.", with puncher holes to the left edges and stapled at the upper left corner. In fine to very fine condition. Accompanied by an autographed letter signed one page, 6c8 inch 30.10.1989 "European Organization for Nuclear Research" letterhead in German, reply letter to Mr. Geyer - concerning the manuscript, written and signed in blue ink "J Steinberger", ith a small tear at the bottom edge - in fine condition
Jack Steinberger was a German-born American physicist noted for his work with neutrinos, the subatomic particles considered to be elementary constituents of matter. He was a recipient of the 1988 Nobel Prize in Physics, along with Leon M. Lederman and Melvin Schwartz, for the discovery of the muon neutrinoIn particle physics, a hadron (/ˈhædrɒn/ (listen); Ancient Greek: ἁδρός, romanized: hadrós; "stout, thick") is a composite subatomic particle made of two or more quarks held together by the strong interaction. They are analogous to molecules that are held together by the electric force. Most of the mass of ordinary matter comes from two hadrons: the proton and the neutron, while most of the mass of the protons and neutrons is in turn due to the binding energy of their constituent quarks, due to the strong force.
Jack Steinberger (born Hans Jakob Steinberger; May 25, 1921 – December 12, 2020) was a German-born American physicist noted for his work with neutrinos, the subatomic particles considered to be elementary constituents of matter. He was a recipient of the 1988 Nobel Prize in Physics, along with Leon M. Lederman and Melvin Schwartz, for the discovery of the muon neutrino. Through his career as an experimental particle physicist, he held positions at the University of California, Berkeley, Columbia University (1950–68), and the CERN (1968–86). He was also a recipient of the United States National Medal of Science in 1988, and the Matteucci Medal from the Italian Academy of Sciences in 1990.
Early life and educationSteinberger was born in the city of Bad Kissingen in Bavaria, Germany, on May 25, 1921 into a Jewish[6] family.[7] The rise of Nazism in Germany, with its open anti-Semitism, prompted his parents, Ludwig Lazarus (a cantor and religious teacher) and Berta May Steinberger,[8][9] to send him out of the country.[7]
Steinberger emigrated to the United States at the age of 13, making the trans-Atlantic trip with his brother Herbert. Jewish charities in the U.S. arranged for Barnett Farroll to care for him as a foster child. Steinberger attended New Trier Township High School, in Winnetka, Illinois.[10] He was reunited with his parents and younger brother in 1938.[8]
Steinberger studied chemical engineering at Armour Institute of Technology (now Illinois Institute of Technology) but left after his scholarship ended to help supplement his family's income.[7] He obtained a bachelor's degree in chemistry from the University of Chicago, in 1942.[7] Shortly thereafter, he joined the Signal Corps at MIT.[11] With the help of the G.I. Bill, he returned to graduate studies at the University of Chicago in 1946, where he studied under Edward Teller and Enrico Fermi.[7][8] His Ph.D. thesis concerned the energy spectrum of electrons emitted in muon decay; his results showed that this was a three-body decay, and implied the participation of two neutral particles in the decay (later identified as the electron (u _{e}) and muon (u _{\mu }) neutrinos) rather than one.[12]
CareerEarly researchAfter receiving his doctorate, Steinberger attended the Institute for Advanced Study in Princeton for a year. In 1949 he published a calculation of the lifetime of the neutral pion,[13] which anticipated the study of anomalies in quantum field theory.[14]
Following Princeton, in 1949, Steinberger went to the Radiation Lab at the University of California at Berkeley, where he performed an experiment which demonstrated the production of neutral pions and their decay to photon pairs. This experiment utilized the 330 MeV synchrotron and the newly invented scintillation counters.[15] Despite this and other achievements, he was asked to leave the Radiation Lab at Berkeley in 1950, due to his refusal to sign the so-called non-Communist Oath.[16][8]
Steinberger accepted a faculty position at Columbia University in 1950. The newly commissioned meson beam at Nevis Labs provided the tool for several important experiments. Measurements of the production cross-section of pions on various nuclear targets showed that the pion has odd parity.[17] A direct measurement of the production of pions on a liquid hydrogen target, then not a common tool, provided the data needed to show that the pion has spin zero. The same target was used to observe the relatively rare decay of neutral pions to a photon, an electron, and a positron. A related experiment measured the mass difference between the charged and neutral pions based on the angular correlation between the neutral pions produced when the negative pion is captured by the proton in the hydrogen nucleus.[18] Other important experiments studied the angular correlation between electron–positron pairs in neutral pion decays, and established the rare decay of a charged pion to an electron and neutrino; the latter required use of a liquid-hydrogen bubble chamber.[19]
Investigations of strange particlesDuring 1954–1955, Steinberger contributed to the development of the bubble chamber with the construction of a 15 cm device for use with the Cosmotron at Brookhaven National Laboratory. The experiment used a pion beam to produce pairs of hadrons with strange quarks to elucidate the puzzling production and decay properties of these particles.[20] In 1956, he used a 30 cm chamber outfitted with three cameras to discover the neutral Sigma hyperon and measure its mass.[21] This observation was important for confirming the existence of the SU(3) flavor symmetry which hypothesizes the existence of the strange quark.[22]
An important characteristic of the weak interaction is its violation of parity symmetry. This characteristic was established through the measurement of the spins and parities of many hyperons. Steinberger and his collaborators contributed several such measurements using large (75 cm) liquid-hydrogen bubble chambers and separated hadron beams at Brookhaven.[23] One example is the measurement of the invariant mass distribution of electron–positron pairs produced in the decay of Sigma-zero hyperons to Lambda-zero hyperons.[24]
Neutrinos and the weak neutral currentIn the 1960s, the emphasis in the study of the weak interaction shifted from strange particles to neutrinos. Leon Lederman, Steinberger and Schwartz built large spark chambers at Nevis Labs and exposed them in 1961 to neutrinos produced in association with muons in the decays of charged pions and kaons. They used the Alternating Gradient Synchrotron (AGS) at Brookhaven, and obtained a number of convincing events in which muons were produced, but no electrons.[25] This result, for which they received the Nobel Prize in 1988, proved the existence of a type of neutrino associated with the muon, distinct from the neutrino produced in beta decay.[26]
Study of CP violationThe CP violation (charge conjugation and parity) was established in the neutral kaon system in 1964. Steinberger recognized that the phenomenological parameter epsilon (ε) which quantifies the degree of CP violation could be measured in interference phenomena (See CP violation). In collaboration with Carlo Rubbia, he performed an experiment while on sabbatical at CERN during 1965 which demonstrated robustly the expected interference effect, and also measured precisely the difference in mass of the short-lived and long-lived neutral kaon masses.[27][28][third-party source needed]
Back in the United States, Steinberger conducted an experiment at Brookhaven to observe CP violation in the semi-leptonic decays of neutral kaons. The charge asymmetry relates directly to the epsilon parameter, which was thereby measured precisely.[29] This experiment also allowed the deduction of the phase of epsilon, and confirmed that CPT is a good symmetry of nature.[30]
CERNIn 1968, Steinberger left Columbia University and accepted a position as a department director at CERN.[31] He constructed an experiment there utilizing multi-wire proportional chambers (MWPC), recently invented by Georges Charpak.[32] The MWPCs, augmented by micro-electronic amplifiers, allowed much larger samples of events to be recorded. Several results for neutral kaons were obtained and published in the early 1970s, including the observation of the rare decay of the neutral kaon to a muon pair, the time dependence of the asymmetry for semi-leptonic decays, and a more-precise measurement of the neutral kaon mass difference. A new era in experimental technique was opened.[33]
These new techniques proved crucial for the first demonstration of direct CP-violation. The NA31 experiment at CERN was built in the early 1980s using the CERN SPS 400 GeV proton synchrotron. As well as banks of MWPCs and a hadron calorimeter, it featured a liquid argon electromagnetic calorimeter with exceptional spatial and energy resolution. NA31 showed that direct CP violation is real.[34]
Steinberger worked on the ALEPH experiment at the Large Electron–Positron Collider (LEP), where he served as the experiment's spokesperson.[35] Among the ALEPH experiment's initial accomplishments was the precise measurement of the number of families of leptons and quarks in the Standard Model through the measurement of the decays of the Z boson.[36]
He retired from CERN in 1986, and went on to become a professor at the Scuola Normale Superiore di Pisa in Italy.[8] He continued his association with the CERN laboratory through his visits into his 90s.[37][7]
Nobel PrizeSteinberger was awarded the Nobel Prize in Physics in 1988, "for the neutrino beam method and the demonstration of the doublet structure of the leptons through the discovery of the muon neutrino".[38] He shared the prize with Leon M. Lederman and Melvin Schwartz; at the time of the research, all three experimenters were at Columbia University.[39]
The experiment used charged pion beams generated with the Alternating Gradient Synchrotron at Brookhaven National Laboratory. The pions decayed to muons which were detected in front of a steel wall; the neutrinos were detected in spark chambers installed behind the wall. The coincidence of muons and neutrinos demonstrated that a second kind of neutrino was created in association with muons. Subsequent experiments proved this neutrino to be distinct from the first kind (electron-type). Steinberger, Lederman and Schwartz published their work in Physical Review Letters in 1962.[25]
He gave his Nobel medal to New Trier High School in Winnetka, Illinois (USA), of which he was an alumnus.[40]
He was also awarded the National Medal of Science in 1988, by the then US president, Ronald Reagan[7] and was the recipient of the Matteucci Medal in 1990, from the Italian Academy of Sciences.[41]
Selected publicationsSteinberger, J. & A. S. Bishop. "The Detection of Artificially Produced Photomesons with Counters", Radiation Laboratory, University of California-Berkeley, United States Department of Energy (through predecessor agency the Atomic Energy Commission), (March 8, 1950).Steinberger, J., W. K. H. Panofsky & J. Steller. "Evidence for the Production of Neutral Mesons by Photons", Radiation Laboratory, University of California-Berkeley, United States Department of Energy (through predecessor agency the Atomic Energy Commission), (April 1950).Panofsky, W. K. H., J. Steinberger & J. Steller. "Further Results on the Production of Neutral Mesons by Photons", Radiation Laboratory, University of California-Berkeley, United States Department of Energy (through predecessor agency the Atomic Energy Commission), (October 1, 1950).Steinberger, J. "Experimental Survey of Strange Particle Decays", Columbia University, Nevis Laboratories, United States Department of Energy (through predecessor agency the Atomic Energy Commission), (June 1964).Steinberger, J. (2005). Learning about particles : 50 privileged years. Berlin: Springer. ISBN 3-540-21329-5. OCLC 56654442.Personal lifeSteinberger's first marriage to Joan Beauregard ended in a divorce, after which he married his former student, biologist Cynthia Alff.[42][8] He had four children, two from each of his marriages.[42] His son Ned Steinberger is the founder of the eponymous company for headless guitars and basses, and his daughter Julia Steinberger is an ecological economist at the University of Lausanne.[43] As an atheist and a humanist, Steinberger was a Humanist Laureate in the International Academy of Humanism.[44][45] In his own words, he is noted to have enjoyed tennis, mountaineering and sailing.[8]
In the 1980s Steinberger resumed relations with his native town Bad Kissingen. He often visited Bad Kissingen after that. The school he had attended there was named Jack-Steinberger-Gymnasium in 2001. In 2006 Steinberger was made honorary citizen of Bad Kissingen. "I feel welcome in Bad Kissingen. This is my hometown and I was raised there. I feel as a German again now" he told the Bavarian broadcasting company Bayerischer Rundfunk in 2013.[46]
He died on December 12, 2020, at his home in Geneva. He was aged 99.[16]
The Nobel Prize in Physics (Swedish: Nobelpriset i fysik) is a yearly award given by the Royal Swedish Academy of Sciences for those who have made the most outstanding contributions for humankind in the field of physics. It is one of the five Nobel Prizes established by the will of Alfred Nobel in 1895 and awarded since 1901, the others being the Nobel Prize in Chemistry, Nobel Prize in Literature, Nobel Peace Prize, and Nobel Prize in Physiology or Medicine. Physics is traditionally the first award presented in the Nobel Prize ceremony.
The first Nobel Prize in Physics was awarded to German physicist Wilhelm Röntgen in recognition of the extraordinary services he rendered by the discovery of X-rays. This award is administered by the Nobel Foundation and is widely regarded as the most prestigious award that a scientist can receive in physics. It is presented in Stockholm at an annual ceremony on 10 December, the anniversary of Nobel's death. As of 2022 a total of 221 individuals have been awarded the prize.[2]
BackgroundAlfred Nobel, in his last will and testament, stated that his wealth should be used to create a series of prizes for those who confer the "greatest benefit on mankind" in the fields of physics, chemistry, peace, physiology or medicine, and literature.[3] Though Nobel wrote several wills during his lifetime, the last one was written a year before he died and was signed at the Swedish-Norwegian Club in Paris on 27 November 1895.[4][5] Nobel bequeathed 94% of his total assets, 31 million Swedish kronor (USD198 million, €176 million in 2016), to establish and endow the five Nobel Prizes.[6] Owing to the level of skepticism surrounding the will, it was not until 26 April 1897 that it was approved by the Storting (Norwegian Parliament).[7][8] The executors of his will were Ragnar Sohlman and Rudolf Lilljequist, who formed the Nobel Foundation to take care of Nobel's fortune and organise the prizes.
The members of the Norwegian Nobel Committee who were to award the Peace Prize were appointed shortly after the will was approved. The other prize-awarding organisations followed: the Karolinska Institutet on 7 June, the Swedish Academy on 9 June, and the Royal Swedish Academy of Sciences on 11 June.[9][10] The Nobel Foundation then established guidelines for awarding the prizes. In 1900, the Nobel Foundation's newly created statutes were promulgated by King Oscar II.[8][11] According to Nobel's will, The Royal Swedish Academy of Sciences would award the Prize in Physics.[11]
Nomination and selection
Three Nobel Laureates in Physics. Front row L-R: Albert A. Michelson (1907 laureate), Albert Einstein (1921 laureate) and Robert A. Millikan (1923 laureate).A maximum of three Nobel laureates and two different works may be selected for the Nobel Prize in Physics.[12] Compared with other Nobel Prizes, the nomination and selection process for the prize in physics is long and rigorous. This is a key reason why it has grown in importance over the years to become the most important prize in Physics.[13]
The Nobel laureates are selected by the Nobel Committee for Physics, a Nobel Committee that consists of five members elected by The Royal Swedish Academy of Sciences. During the first stage that begins in September, a group of about 3,000 selected university professors, Nobel Laureates in Physics and Chemistry, and others are sent confidential nomination forms. The completed forms must arrive at the Nobel Committee by 31 January of the following year. The nominees are scrutinized and discussed by experts and are narrowed to approximately fifteen names. The committee submits a report with recommendations on the final candidates to the Academy, where, in the Physics Class, it is further discussed. The Academy then makes the final selection of the Laureates in Physics by a majority vote.[14]
The names of the nominees are never publicly announced, and neither are they told that they have been considered for the Prize. Nomination records are sealed for fifty years.[15] While posthumous nominations are not permitted, awards can be made if the individual died in the months between the decision of the committee (typically in October) and the ceremony in December. Prior to 1974, posthumous awards were permitted if the candidate had died after being nominated.[16]
The rules for the Nobel Prize in Physics require that the significance of achievements being recognized has been "tested by time". In practice, that means that the lag between the discovery and the award is typically on the order of 20 years and can be much longer. For example, half of the 1983 Nobel Prize in Physics was awarded to Subrahmanyan Chandrasekhar for his work on stellar structure and evolution that was done during the 1930s. As a downside of this tested-by-time rule, not all scientists live long enough for their work to be recognized. Some important scientific discoveries are never considered for a prize, as the discoverers die by the time the impact of their work is appreciated.[17][18]
PrizesA Physics Nobel Prize laureate is awarded a gold medal, a diploma bearing a citation, and a sum of money.[19]
MedalsThe Nobel Prize medals, minted by Myntverket[20] in Sweden and the Mint of Norway since 1902, are registered trademarks of the Nobel Foundation. Each medal has an image of Alfred Nobel in left profile on the obverse. The Nobel Prize medals for physics, chemistry, physiology or medicine, and literature have identical obverses, showing the image of Alfred Nobel and the years of his birth and death (1833–1896). Nobel's portrait also appears on the obverse of the Nobel Peace Prize medal and the Medal for the Prize in Economics, but with a slightly different design.[21][22] The image on the reverse of a medal varies according to the institution awarding the prize. The reverse sides of the Nobel Prize medals for chemistry and physics share the same design of the Goddess of Nature, whose veil is held up by the Genius of Science. These medals, along with those for Physiology/Medicine and Literature, were designed by Erik Lindberg in 1902.[23]
Diplomas
1903 Nobel Prize diploma, awarded to Marie Curie and Pierre CurieNobel laureates receive a diploma directly from the hands of the King of Sweden. Each diploma is uniquely designed by the prize-awarding institutions for the laureate who receives it.[24] The diploma contains a picture with the name of the laureate and a citation explaining their accomplishments.[24]
Award moneyAt the awards ceremony, the laureate is given a document indicating the award sum. The amount of the cash award may differ from year to year, based on the funding available from the Nobel Foundation. For example, in 2009 the total cash awarded was 10 million SEK (US$1.4 million),[25] but in 2012 following the Great Recession, the amount was 8 million Swedish Kronor, or US$1.1 million.[26] If there are two laureates in a particular category, the award grant is divided equally between the recipients, but if there are three, the awarding committee may opt to divide the grant equally, or award half to one recipient and a quarter to each of the two others.[27][28][29][30]
CeremonyThe committee and institution serving as the selection board for the prize typically announce the names of the laureates during the first week of October. The prize is then awarded at formal ceremonies held annually in Stockholm Concert Hall on 10 December, the anniversary of Nobel's death. The laureates receive a diploma, a medal and a document confirming the prize amount.[31]
The muon neutrino is an elementary particle which has the symbolνμ and zero electric charge. Together with the muon it forms the second generation of leptons, hence the name muon neutrino. It was discovered in 1962 by Leon Lederman, Melvin Schwartz and Jack Steinberger. The discovery was rewarded with the 1988 Nobel Prize in Physics.
DiscoveryThe muon neutrino or "neutretto" was hypothesized to exist by a number of physicists in the 1940s.[1] The first paper on it may be Shoichi Sakata and Takesi Inoue's two-meson theory of 1942, which also involved two neutrinos.[2][3] In 1962 Leon M. Lederman, Melvin Schwartz and Jack Steinberger proved the existence of the muon neutrino in an experiment at the Brookhaven National Laboratory.[4] This earned them the 1988 Nobel Prize.[5]
SpeedMain article: Faster-than-light neutrino anomalyIn September 2011 OPERA researchers reported that muon neutrinos were apparently traveling at faster than light speed. This result was confirmed again in a second experiment in November 2011. These results were viewed skeptically by the scientific community at large, and more experiments investigated the phenomenon. In March 2012 the ICARUS team published results directly contradicting the results of OPERA.[6]
Later, in July 2012, the apparent anomalous super-luminous propagation of neutrinos was traced to a faulty element of the fibre optic timing system in Gran-Sasso. After it was corrected the neutrinos appeared to travel with the speed of light within the errors of the experiment.[7]
was born in Bad Kissingen (Franconia) in 1921. At that time my father, Ludwig, was 45 years old. He was one of twelve children of a rural ‘Viehhändler’ (small-time cattle dealer). Since the age of eighteen he had been cantor and religious teacher for the little Jewish community, a job he still held when he emigrated in 1938. He had been a bachelor until he returned from four years of service in the German Army in the first World War. My mother was born in Nuremberg to a hop merchant, and was fifteen years the younger. Unusual for her time, she had the benefit of a college education and supplemented the meagre income with English and French lessons, mostly to the tourists which provided the economy of the spa. The childhood I shared with my two brothers was simple; Germany was living through the post-war depression.
Things took a dramatic turn when I was entering my teens. I remember Nazi election propaganda posters showing a hateful Jewish face with crooked nose, and the inscription “Die Juden sind unser Ungluck”, as well as torchlight parades of SA storm troops singing “Wenn’s Juden Blut vom Messer fliesst, dann geht’s noch mal so gut”. In 1933, the Nazis came to power and the more systematic persecution of the Jews followed quickly. Laws were enacted which excluded Jewish children from higher education in public schools. When, in 1934, the American Jewish charities offered to find homes for 300 German refugee children, my father applied for my older brother and myself. We were on the SS Washington, bound for New York, Christmas 1934.
I owe the deepest gratitude to Barnett Faroll, the owner of a grain brokerage house on the Chicago Board of Trade, who took me into his house, parented my high-school education, and made it possible also for my parents and younger brother to come in 1938 and so to escape the holocaust. New Trier Township High School on the well-to-do Chicago North Shore, enjoyed a national reputation, and, with a swimming pool, athletic fields, cafeteria, as well as excellent teachers, offered horizons unimaginable to the young emigrant from a small German town.
The reunited family settled down in Chicago. We were helped to acquire a small delicatessen store which was the basis of a very marginal income, but we were used to a simple life, so this was no problem. I was able to continue my education for two years at the Armour Institute of Technology (now the Illinois Institute of Technology) where I studied chemical engineering. I was a good student, but these were the hard times of the depression, my scholarship came to an end, and it was necessary to work to supplement the family income.
The experience of trying to find a job as a twenty-year-old boy without connections was the most depressing I was ever to face. I tried to find any job in a chemical laboratory: I would present myself, fill out forms, and have the door closed hopelessly behind me. Finally through a benefactor of my older brother, I was accepted to wash chemical apparatus in a pharmaceutical laboratory, G.D. Searl and Co., at eighteen dollars a week. In the evenings I studied chemistry at the University of Chicago, the weekends I helped in the family store.
The next year, with the help of a scholarship from the University of Chicago, I could again attend day classes, so that in 1942 I could finish an undergraduate degree in chemistry.
On 7 December 1941, Japan attacked the United States at Pearl Harbor. I joined the Army and was sent to the MIT radiation laboratory after a few months of introduction to electromagnetic wave theory in a special course, given for Army personnel at the University of Chicago. My only previous contact with physics had been the sophomore introductory course at Armour. The radiation laboratory was engaged in the development of radar bomb sights; I was assigned to the antenna group. Among the outstanding physicists in the laboratory were Ed Purcell and Julian Schwinger. The two years there offered me the opportunity to take some basic courses in physics.
After Germany surrendered in 1945, I spent some months on active duty in the Army, but was released after the Japanese surrender, to continue my studies at the University of Chicago. It was a wonderful atmosphere, both between professors and students and also among the students. The professors to whom I owe the greatest gratitude are Enrico Fermi, W. Zachariasen, Edward Teller and Gregor Wentzel. The courses of Fermi were gems of simplicity and clarity and he made a great effort to help us become good physicists also outside the regular class-room work, by arranging evening discussions on a widespread series of topics, where he also showed us how to solve problems. Fellow students included Yang, Lee, Goldberger, Rosenbluth, Garwin, Chamberlain, Wolfenstein and Chew. There was a marvellous collaboration, and I feel I learned as much from these fellow students as from the professors.
I would have preferred to do a theoretical thesis, but nothing within reach of my capabilities seemed to offer itself. Fermi then asked me to look into a problem raised in an experiment by Rossi and Sands on stopping cosmic-ray muons. They did not find the expected number of decays. After correcting for geometrical losses there was still a missing factor of two, and I suggested to Sands that this might be due to the fact that the decay electron had less energy than expected in the two-body decay, and that one might test this experimentally. When this idea was not followed, Fermi suggested that I do the experiment, instead of waiting for a theoretical topic to surface. The cosmic-ray experiment required less than a year from its conception to its conclusion, in the end of the summer of 1948. It showed that the muon’s is a three-body decay, probably into an electron and two neutrinos, and helped lay the experimental foundation for the concept of a universal weak interaction.
There followed an interlude to try theory again at the Institute for Advanced Study in Princeton, where Oppenheimer had become director. It was a frustrating year: I was no match for Dyson and other young theoreticians assembled there. Towards the end I managed to find a piece of work I could do, on the decay of mesons via intermediate nucleons. I still remember how happy Oppenheimer was to see me come up with something, at last.
In 1949, Gian Carlo Wick, with whom I had done some work on the scattering of polarized neutrons in magnetized iron while still a graduate student at Chicago University, invited me to be his assistant at the University of California in Berkeley. There the experimental possibilities in the Radiation Laboratory, created by E.O. Lawrence, were so great that I reverted easily to my wild state, that is experimentation. During the year there, I had the magnificent opportunity of working on the just completed electron synchrotron of Ed McMillan. It enabled me to do the first experiments on the photoproduction of pions (with A.S. Bishop) to establish the existence of neutral pions (with W.K.H. Panofsky and J. Stellar) as well as to measure the pion mean life (with O. Chamberlain, R.F. Mozley and C. Weigand).
I survived only a year in Berkeley, partly because I declined to sign the anticommunist loyalty oath, and moved on to Columbia University in the summer of 1950. At its Nevis Laboratory, Columbia had just completed a 380 MeV cyclotron; this, for the first time, offered the possibility of experimenting with beams of T mesons. In the next years I exploited these beams to determine the spins and parities of charged and neutral pions, to measure the pi– pi0 mass difference and to study the scattering of charged pions. This work leaned heavily on the collaboration of Profs. D. Bodansky and A.M. Sachs, as well as of several Ph.D. students: R. Durbin, H. Loar, P. Lindenfeld, W. Chinowsky and S. Lokanathan.
These experiments all utilized small scintillator counters. In the early fifties, the bubble-chamber technique was discovered by Don Glaser, and in 1954 three graduate students, J. Leitner, N.P. Samios and M. Schwartz, and myself began to study this technique which had not as yet been exploited to do physics. Our first effort was a 10 cm diameter propane chamber. We made one substantial contribution to the technique, that was the realization of a fast recompression (within ~10 ms), so that the bubbles were recompressed before they could grow large and move to the top. This permitted chamber operation at a useful cycling rate. The first bubble-chamber paper to be published was from our experiment at the newly built Brookhaven Cosmotron, using a 15 cm propane chamber without magnetic field. It yielded a number of results on the properties of the new unstable (strange) particles at a previously unattainable level, and so dramatically demonstrated the power of the new technique which was to dominate particle physics for the next dozen years. Only a few months later we published our findings on three events of the type Sigma0-> Delta0 + gamma, which demonstrated the existence of the Sigma0 hyperon and gave a measure of its mass. This experiment used a new propane chamber, eight times larger in volume, and with a magnetic field. This chamber also introduced the use of more than two stereo cameras, a development which is crucial for the rapid, computerized analysis of events, and has been incorporated into all subsequent bubble chambers.
In the decade which followed, the same collaborators, together with Profs. Plano, Baltay, Franzini, Colley and Prodell, and a number of new students, constructed three more bubble chambers: a 12″ H2 chamber as well as 30″ propane and H2 chambers, developed the analysis techniques, and performed a series of experiments to clarify the properties of the new particles. The experiments I remember with the most pleasure are:
– the demonstration of parity violation in D decay, 1957;– the demonstration of the ß decay of the pion, 1958;– the determination of the p0 parity on the basis of angular correlation in the double internal conversion of the g rays, 1962;– the determination of the w and j decay widths (lifetimes), 1962;– the determination of the S0 – D0 relative parity, 1963;– the demonstration of the validity of the DS = DQ rule in K0 and in hyperon decays, 1964.
This long chain of bubble-chamber experiments, in which I also enjoyed and appreciated the collaboration of two Italian groups, the Bologna group of G. Puppi and the Pisa group of M. Conversi, was interrupted in 1961, in order to perform, at the suggestion of Mel Schwartz, and with G. Danby, J.M. Gaillard, D. Goulianos, L. Lederman and N. Mistri, the first experiment using a high-energy neutrino beam now recognized by the Nobel Prize, and described in the paper of M. Schwartz.
In 1964, CP violation was discovered by Christensen, Cronin, Fitch and Turlay. Soon after I found myself on sabbatical leave at CERN, and proposed, together with Rubbia and others, to look for the interference between K0s and K0L amplitudes in the time dependence of K0 decay. Such interference was expected in the CP violation explanation of the results of Christensen et al., but not in other explanations which had also been proposed. The experiment was successful, and marked the beginning of a set of experiments to learn more about CP violation, which was to last a decade. The next result was the observation of the small, CP-violating, charge asymmetry in K0L leptonic decay, in 1966. Measurement of the time dependence of this charge asymmetry, following a regenerator, permitted a determination of the regeneration phase; this, together with the earlier interference experiments, yielded, for the first time, the CP-violating phase jh+ – and, in consequence, as well as the observed magnitudes of the CP-violating amplitudes in the two-pion and the leptonic decays, certain checks of the superweak model. The same experiment also gave a more sensitive check of the DS = DQ rule, an ingredient of the present Standard Model.
In 1968, I joined CERN. Charpak had just invented proportional wire chambers, and this development offered a much more powerful way to study the K0 decay to which I had become addicted. Two identical detectors were constructed, one at CERN together with Filthuth, Kleinknecht, Wahl, and others, and one at Columbia together with Christensen, Nygren, Carithers and students. The Columbia beam was long, and therefore contained no Ks but only KL, the CERN beam was short, and therefore contained a mixture of Ks and KL. It was contaminated by a large flux of L0, and so was also a hyperon beam, permitting the first measurements of L0 cross-sections as well as the Coulomb excitation of L0 to S0, a difficult and interesting experiment carried out chicfly by Steffen and Dydak. The most important result to come from the Columbia experiment was the observation of the rare decay KL -> µ+µ– with a branching ratio compatible with theoretical predictions based on unitarity. Previously, a Berkeley experiment had searched in vain for this decay and had claimed an upper limit in violation of unitarity. Since unitarity is fundamental to field theory, this result had a certain importance.
The CERN experiment, which extended until 1976, produced a series of precise measurements on the interference of Ks and KL in the two-pion and leptonic decay modes, thus leading us to obtain highly precise results on the CP-violating parameters in K0 decay. I believe the experiment was beautiful, and take some pride in it, but the results were all in agreement with the superweak model and so did little towards understanding the origin of CP violation.
In 1972, the K0 collaboration of CERN, Dortmund and Heidelberg was joined by a group from Saclay, under R. Turlay, to study the possibilities for a neutrino experiment at the CERN SPS then under construction. The CDHS detector, a modular array of magnetized iron disks, scintillation counters and drift chambers, 3.75 m in diameter, 20 m long, and weighing 1200 t, was designed, constructed, and exposed to different neutrino beams at the SPS during the period 1977 to 1983. It provided a large body of data on the charged-current and neutral-current inclusive reactions in iron, which permitted first of all the clearing away of a number of incorrect results, e.g. the “high-y anomaly” produced at Fermilab, allowed the first precise and correct determination of the Weinberg angle, demonstrated the existence of right-handed neutral currents, provided measurements of the structure functions which gave quantitative support to the quark constituent model of the nucleon, and, through the Q2 evolution of the structure functions, gave quantitative support to QCD. The study of multimuon events gave quantitative support to the GIM model of the Cabibbo current through its predictions on charm production.
In the CDHS experiment we were about thirty physicists. Since 1983, I have been spokesman for a collaboration of 400 physicists engaged in the design and construction of a detector for the 100 + 100 GeV e+e– Collider, LEP, to be ready at CERN in the beginning of 1989. In the meantime I had also helped to design an experiment to compare CP violation in the charged and neutral two-pion decay of the K0L. This experiment was the first to show “direct” CP violation, an important step towards the understanding of CP violation.
In 1986, I retired from CERN and became part-time Professor at the Scuola Normale Superiore in Pisa. However, my chief activity continues as before in my research at CERN.
I am married to Cynthia Alff, my former student and now biologist, and we have two marvellous children, Julia, 14 years old, and John, 11 years old. From an earlier marriage to Joan Beauregard, there are two fine sons, Joseph Ludwig and Richard Ned.
I play the flute, unfortunately not very well, and have enjoyed tennis, mountaineering and sailing, passionately.
From Nobel Lectures, Physics 1981-1990, Editor-in-Charge Tore Frängsmyr, Editor Gösta Ekspong, World Scientific Publishing Co., Singapore, 1993This autobiography/biography was written at the time of the award and first published in the book series Les Prix Nobel. It was later edited and republished in Nobel Lectures. To cite this document, always state the source as shown above.
Copyright © The Nobel Foundation 1988Addendum, June 2005In 1988, I was the spokesman of a collaboration of about 350 physicists, preparing the detector we called ALEPH, which we had started to plan in 1981, for the CERN electron-positron collider then under construction called LEP, and which started to operate in 1989. Altogether, about fifteen hundred physicists participated, using four such detectors. LEP results dominated CERN physics, perhaps the world’s, for a dozen or more years, with crucial, precise measurements, which confirmed the Standard Model of the unified electro-weak and strong interactions. The physics scene had changed a lot since the time of my thesis experiment in 1948, which I could do quite alone. For some time I could help, as manager, but also contributing to the detector design and the physics analysis. This came to an end in 1995, partly because I had no new ideas on the physics we might learn, and partly because the challenges became more and more technical, especially in the use of computers, and I could not compete with the younger generation.
Since that time I have enjoyed learning cosmology and astrophysics, and following its progress. This has given me much satisfaction: on the one hand it involved having to learn some basic physics new to me, physics important to cosmology but unimportant in particle physics, such as general relativity and hydrodynamics, on the other hand these have been spectacular years in astrophysics, with the discovery in 1992, and continually improving observational results, of the inhomogeneities of the cosmic microwave background radiation, which give a totally new map of the universe, at a much earlier time than stars or galaxies, much simpler and therefore much easier to learn from, and more precisely. I still come to CERN, the 10 km on my bicycle, every day and sometimes enjoy trying to learn something new.
Jack Steinberger, who shared the 1988 Nobel Prize in Physics for expanding understanding of the ghostly neutrino, a staggeringly ubiquitous subatomic particle, died on Saturday at his home in Geneva. He was 99.
His wife, Cynthia Alff, confirmed the death.
The ancient Greeks proposed that there was one invisible, indivisible unit of matter: the atom. But modern physics has found more than 100 smaller entities lurking within atoms, and observations of their dizzying interactions compose the Standard Model of what is now taken to be the order of the universe.
The neutrino’s existence was first proposed in 1931, to fill holes in a theory about the makeup of the universe, but finding one proved maddeningly difficult. It has no electrical charge, travels at nearly the speed of light and has almost no mass. Each second, trillions of neutrinos pass unimpeded through every human being. Not until 1956 — when fission nuclear reactors and instruments to detect particles flying out from them were developed — was one detected.
ImageFrom left, Leon E. Lederman, Dr. Steinberger and Melvin Schwartz in 1988, the year they shared the Nobel Prize in Physics.From left, Leon E. Lederman, Dr. Steinberger and Melvin Schwartz in 1988, the year they shared the Nobel Prize in Physics.Credit...CERN
Six years later, Dr. Steinberger joined with two fellow Columbia University physicists, Melvin Schwartz and Leon M. Lederman, to show that two types of neutrinos existed. Just as significant, they devised a method to produce a beam composed of vast numbers of neutrinos at very high energies. The beam could be used to study one of the basic forces of nature: the weak interaction, virtually the only influence to which neutrinos respond.
ADVERTISEMENT
Continue reading the main storyThis weak interaction is one of the four fundamental interactions of nature, along with the strong interaction, which binds an atom’s nucleus together, as well as electromagnetism and gravitation. It is responsible for the radioactive decay and nuclear fusion of subatomic particles, and is called weak because its strength is surpassed by both the strong force and electromagnetism. Gravitation is weaker still.
You have 1 free article remaining.Subscribe to The TimesIn bestowing the physics prize on the three men, the Nobel awards committee said they had “opened entirely new opportunities for research into the innermost structure and dynamics of matter.”
An experiment they conducted in 1961-62 used a new half-mile-long accelerator at the Brookhaven National Laboratory on Long Island to fire bursts of protons into a piece of beryllium metal. The collisions tore apart the beryllium nuclei, generating a flood of evanescent particles that fell apart in a cascade of debris.
These particles were then hurled into a 40-foot-thick wall of steel, which stopped all of them except neutrinos. The neutrino beam was then sent through 90 one-inch-thick aluminum plates. What came out on the other side was the team’s discovery: that there were two kinds of neutrinos. (Other researchers later discovered a third type.) And because neutrinos are affected only by the weak interactions, the neutrino beam allowed particle physicists to investigate such interactions. The long-mysterious neutrino became a research tool.
Editors’ Picks
Strep Is on the Rise. Here’s How to Minimize Your Risk.
Religious Pop Star Singing of ‘God and Faith’ Wins Over Secular Israel
Getting Married in a Nap DressContinue reading the main storyADVERTISEMENT
Continue reading the main storyNormally, an experiment at the Brookhaven accelerator — the world’s largest at the time — was completed in a few hours. The neutrino experiment consumed 800 hours over eight months, a measure of the importance attached to the project. A year after it was finished, the European Council for Nuclear Research, known as CERN, confirmed the results.
Hans Jakob Steinberger was born on May 25, 1921, in Bad Kissingen in Bavaria, Germany, one of three sons of Ludwig and Berta Steinberger. His father was a cantor and religious teacher to the town’s small Jewish community; his college-educated mother supplemented the family income by giving English and French lessons.
With the rise of the Nazis and the enactment of laws barring Jewish children from attending public schools and from seeking higher education, his parents arranged for him and his older brother to go to the United States with the help of the American Jewish charities, which had offered to find homes for 300 German refugee children.
Soon, Dr. Steinberger wrote in a biographical sketch for the Nobel Foundation, “we were on the S.S. Washington, bound for New York, Christmas 1934.”
The brothers were placed in separate but nearby foster homes in the Chicago area. Jack settled into the home of a wealthy grain broker named Barnett Faroll, who several years later arranged for the boys’ parents and younger brother to join them in Chicago, rescuing them from the Holocaust, Dr. Steinberger wrote.
Jack Steinberger, who has died aged 99, was one of the three winners of the Nobel prize for physics in 1988 for their work with neutrinos and the discovery of the muon-neutrino. This research did much to advance understanding of fundamental particles.
The reality of the ghostly neutrino was not confirmed experimentally until 1956, but back in 1948, working at the University of Chicago, Steinberger had first given indirect hints of its presence in his measurement of decays of the muon – a heavy sibling of the electron. He showed that when a muon converts into an electron, two very light, possibly massless, electrically neutral particles are also produced. These, it was later shown, are neutrinos.
With his demonstration that the muon did not decay by the more direct route of radiating a photon, Steinberger had unwittingly also given the first clue that a muon is not simply a heavy version of the electron but has some intrinsic “muon flavour”. What that “flavour” is remains a mystery, but it has become a staple of modern particle physics theory.
Soon theorists suspected that the two neutrinos produced in the muon decay could not be identical. The idea emerged that there are two flavours of neutrino: the “electron-neutrino” with affinity for the electron, and its sibling the “muon-neutrino”, analogously paired with the muon. The experimental proof of this fundamental key to the pattern of fundamental particles came in 1962 thanks to Steinberger and his colleagues Melvin Schwartz and Leon Lederman.
At Brookhaven National Laboratory on Long Island, New York, the trio developed techniques for producing intense beams of high energy neutrinos. At high energies, the chance of neutrinos interacting with material targets increases to a level that makes them experimentally viable. Their breakthrough inspired decades of experimental work involving neutrinos, and their first experiment with high energy neutrinos confirmed the distinctive identities of the electron-neutrino and muon-neutrino.
In essence, there were two ways of producing neutrinos, one where they emerge in association with a muon, another where they come with an electron. According to the theory, the former are muon-neutrinos, the latter electron-neutrinos. What Steinberger and his colleagues discovered was that when beams of the former variety – muon-neutrinos – hit targets and picked up electric charge, the charge was invariably carried by a muon, whereas in the other case, the charge was carried by an electron.
This confirmed that neutrinos carry a memory of their birth, some metaphorical DNA that is passed on to progeny in subsequent interactions. This DNA analogue is known as “flavour” and became a key property in the modern Standard Model of fundamental particles.
He was born Hans Jakob Steinberger in Bad Kissingen, a Bavarian spa settlement in Germany, the son of May and Ludwig Steinberger. His father, a teacher from the town’s small Jewish community, had been a veteran of the German army in the first world war, but with the rise of the Nazis the Steinberger family had no future in their country. In 1934 Hans and his older brother headed for the US, sponsored by an American organisation that had volunteered to bring 300 Jewish children to the country. The rest of the family joined them a year later.
His parents subsequently ran a delicatessen in Winnetka, Illinois, where Jack – as he was known after his arrival in the US – attended New Trier high school and what was then the Armour Institute of Technology, Chicago, before entering the University of Chicago. There he gained a chemistry degree and made a computation of the lifetime of the electrically neutral pi meson, which later played a significant role in the development of theoretical particle physics. Nonetheless, Steinberger felt he was not good enough to shine at theory, and instead focused on experiments. During the second world war he worked on the development of radar in the radiation laboratory at the Massachusetts Institute of Technology, before returning to Chicago to study physics under Enrico Fermi, gaining his doctorate in 1948.
He then went to the University of California at Berkeley for a year but left because, though not a communist, he refused on principle to sign a loyalty oath attesting to the fact. He moved to Columbia University, New York, joining the faculty in 1950 and eventually becoming a full professor there. He left Columbia to take up a post at the European centre for particle physics, Cern, in Geneva, in 1968.
Jack Steinberger, left, in conversation with fellow physicist Lev Landau of the Soviet Union, right, at a scientific conference on high energy particles in 1956.Jack Steinberger, left, in conversation with fellow physicist Lev Landau of the Soviet Union, right, at a scientific conference on high energy particles in 1956. Photograph: Sovfoto/Universal Images Group/Rex/ShutterstockIn 1988 Steinberger was one of the prime movers behind the Aleph collaboration of more than 300 physicists working at Cern’s electron-positron collider, LEP, then under construction. LEP was specially designed to produce the massive Z boson, which is a key agent in the weak nuclear force and is also a portal into studying neutrinos. From the precision measurements of the Z boson’s properties came proof that there are not two but three – and no more than three – varieties of lightweight neutrinos. In so doing, particle physics established a modern analogue of Dmitri Mendeleev’s periodic table of the elements, whose capstone, the Higgs boson, was discovered in 2012.
Steinberger never forgot his reception in the US as a refugee. He donated his Nobel prize medal to his old school, noting that its “good beginning was one of several important privileges in my life”.
He remained active in research and was a regular presence at Cern lectures into his 90s.
His first marriage, to Joan Beauregard, ended in divorce, after which he married Cynthia Alff, a biologist. He is survived by his four children, two from each marriage.
Jack Steinberger, physicist, born 25 May 1921; died 12 December 2020
This article was amended on 22 January 2021 to correct details of Jack Steinberger’s early years.
I hope you appreciated this article. Before you move on, I was hoping you would consider taking the step of supporting the Guardian’s journalism.
From Elon Musk to Rupert Murdoch, a small number of billionaire owners have a powerful hold on so much of the information that reaches the public about what’s happening in the world. The Guardian is different. We have no billionaire owner or shareholders to consider. Our journalism is produced to serve the public interest – not profit motives.
And we avoid the trap that befalls much US media – the tendency, born of a desire to please all sides, to engage in false equivalence in the name of neutrality. While fairness guides everything we do, we know there is a right and a wrong position in the fight against racism and for reproductive justice. When we report on issues like the climate crisis, we’re not afraid to name who is responsible. And as a global news organization, we’re able to provide a fresh, outsider perspective on US politics – one so often missing from the insular American media bubble.
Around the world, readers can access the Guardian’s paywall-free journalism because of our unique reader-supported model. That’s because of people like you. Our readers keep us independent, beholden to no outside influence and accessible to everyone – whether they can afford to pay for news, or not.The Large Hadron Collider (LHC) is the world's largest and highest-energy particle collider.[1][2] It was built by the European Organization for Nuclear Research (CERN) between 1998 and 2008 in collaboration with over 10,000 scientists and hundreds of universities and laboratories, as well as more than 100 countries.[3] It lies in a tunnel 27 kilometres (17 mi) in circumference and as deep as 175 metres (574 ft) beneath the France–Switzerland border near Geneva.
The first collisions were achieved in 2010 at an energy of 3.5 teraelectronvolts (TeV) per beam, about four times the previous world record.[4][5] The discovery of the Higgs boson at the LHC was announced in 2012. Between 2013 and 2015, the LHC was shut down and upgraded; after those upgrades it reached 6.8 TeV per beam (13.6 TeV total collision energy).[6][7][8][9] At the end of 2018, it was shut down for three years for further upgrades.
The collider has four crossing points where the accelerated particles collide. Nine detectors,[10] each designed to detect different phenomena, are positioned around the crossing points. The LHC primarily collides proton beams, but it can also accelerate beams of heavy ions: lead–lead collisions and proton–lead collisions are typically performed for one month a year.
The LHC's goal is to allow physicists to test the predictions of different theories of particle physics, including measuring the properties of the Higgs boson,[11] searching for the large family of new particles predicted by supersymmetric theories,[12] and other unresolved questions in particle physics.
BackgroundThe term hadron refers to subatomic composite particles composed of quarks held together by the strong force (analogous to the way that atoms and molecules are held together by the electromagnetic force).[13] The best-known hadrons are the baryons such as protons and neutrons; hadrons also include mesons such as the pion and kaon, which were discovered during cosmic ray experiments in the late 1940s and early 1950s.[14]
A collider is a type of a particle accelerator which brings two opposing particle beams together such that the particles collide. In particle physics, colliders, though harder to construct, are a powerful research tool because they reach a much higher center of mass energy than fixed target setups. [1] Analysis of the byproducts of these collisions gives scientists good evidence of the structure of the subatomic world and the laws of nature governing it. Many of these byproducts are produced only by high-energy collisions, and they decay after very short periods of time. Thus many of them are hard or nearly impossible to study in other ways.[15]
PurposeMany physicists hope that the Large Hadron Collider will help answer some of the fundamental open questions in physics, which concern the basic laws governing the interactions and forces among the elementary objects, the deep structure of space and time, and in particular the interrelation between quantum mechanics and general relativity.[16]
Data is also needed from high-energy particle experiments to suggest which versions of current scientific models are more likely to be correct – in particular to choose between the Standard Model and Higgsless model and to validate their predictions and allow further theoretical development.
Issues explored by LHC collisions include:[17][18]
Is the mass of elementary particles being generated by the Higgs mechanism via electroweak symmetry breaking?[19] It was expected that the collider experiments will either demonstrate or rule out the existence of the elusive Higgs boson, thereby allowing physicists to consider whether the Standard Model or its Higgsless alternatives are more likely to be correct.[20][21]Is supersymmetry, an extension of the Standard Model and Poincaré symmetry, realized in nature, implying that all known particles have supersymmetric partners?[22][23][24]Are there extra dimensions,[25] as predicted by various models based on string theory, and can we detect them?[26]What is the nature of the dark matter that appears to account for 27% of the mass–energy of the universe?Other open questions that may be explored using high-energy particle collisions:
It is already known that electromagnetism and the weak nuclear force are different manifestations of a single force called the electroweak force. The LHC may clarify whether the electroweak force and the strong nuclear force are similarly just different manifestations of one universal unified force, as predicted by various Grand Unification Theories.Why is the fourth fundamental force (gravity) so many orders of magnitude weaker than the other three fundamental forces? See also Hierarchy problem.Are there additional sources of quark flavour mixing, beyond those already present within the Standard Model?Why are there apparent violations of the symmetry between matter and antimatter? See also CP violation.What are the nature and properties of quark–gluon plasma, thought to have existed in the early universe and in certain compact and strange astronomical objects today? This will be investigated by heavy ion collisions, mainly in ALICE, but also in CMS, ATLAS and LHCb. First observed in 2010, findings published in 2012 confirmed the phenomenon of jet quenching in heavy-ion collisions.[27][28][29]DesignThe collider is contained in a circular tunnel, with a circumference of 26.7 kilometres (16.6 mi), at a depth ranging from 50 to 175 metres (164 to 574 ft) underground. The variation in depth was deliberate, to reduce the amount of tunnel that lies under the Jura Mountains to avoid having to excavate a vertical access shaft there. A tunnel was chosen to avoid having to purchase expensive land on the surface, which would also have an impact on the landscape and to take advantage of the shielding against background radiation that the earth's crust provides.[30]Map of the Large Hadron Collider at CERNThe 3.8-metre (12 ft) wide concrete-lined tunnel, constructed between 1983 and 1988, was formerly used to house the Large Electron–Positron Collider.[31] The tunnel crosses the border between Switzerland and France at four points, with most of it in France. Surface buildings hold ancillary equipment such as compressors, ventilation equipment, control electronics and refrigeration plants.Superconducting quadrupole electromagnets are used to direct the beams to four intersection points, where interactions between accelerated protons will take place.The collider tunnel contains two adjacent parallel beamlines (or beam pipes) each containing a beam, which travel in opposite directions around the ring. The beams intersect at four points around the ring, which is where the particle collisions take place. Some 1,232 dipole magnets keep the beams on their circular path (see image[32]), while an additional 392 quadrupole magnets are used to keep the beams focused, with stronger quadrupole magnets close to the intersection points in order to maximize the chances of interaction where the two beams cross. Magnets of higher multipole orders are used to correct smaller imperfections in the field geometry. In total, about 10,000 superconducting magnets are installed, with the dipole magnets having a mass of over 27 tonnes.[33] Approximately 96 tonnes of superfluid helium-4 is needed to keep the magnets, made of copper-clad niobium-titanium, at their operating temperature of 1.9 K (−271.25 °C), making the LHC the largest cryogenic facility in the world at liquid helium temperature. LHC uses 470 tonnes of Nb–Ti superconductor.[34]
During LHC operations, the CERN site draws roughly 200 MW of electrical power from the French electrical grid, which, for comparison, is about one-third the energy consumption of the city of Geneva; the LHC accelerator and detectors draw about 120 MW thereof.[35] Each day of its operation generates 140 terabytes of data.[36]
When running an energy of 6.5 TeV per proton,[37] once or twice a day, as the protons are accelerated from 450 GeV to 6.5 TeV, the field of the superconducting dipole magnets is increased from 0.54 to 7.7 Tesla Referrals (T). The protons each have an energy of 6.5 TeV, giving a total collision energy of 13 TeV. At this energy, the protons have a Lorentz factor of about 6,930 and move at about 0.999999990 c, or about 3.1 m/s (11 km/h) slower than the speed of light (c). It takes less than 90 microseconds (μs) for a proton to travel 26.7 km around the main ring. This results in 11,245 revolutions per second for protons whether the particles are at low or high energy in the main ring, since the speed difference between these energies is beyond the fifth decimal.[38]
Rather than having continuous beams, the protons are bunched together, into up to 2,808 bunches, with 115 billion protons in each bunch so that interactions between the two beams take place at discrete intervals, mainly 25 nanoseconds (ns) apart, providing a bunch collision rate of 40 MHz. It was operated with fewer bunches in the first years. The design luminosity of the LHC is 1034 cm−2s−1,[39] which was first reached in June 2016.[40] By 2017, twice this value was achieved.[41]The LHC protons originate from the small red hydrogen tank.Before being injected into the main accelerator, the particles are prepared by a series of systems that successively increase their energy. The first system is the linear particle accelerator Linac4 generating 160 MeV negative hydrogen ions (H− ions), which feeds the Proton Synchrotron Booster (PSB). There, both electrons are stripped from the hydrogen ions leaving only the nucleus containing one proton. Protons are then accelerated to 2 GeV and injected into the Proton Synchrotron (PS), where they are accelerated to 26 GeV. Finally, the Super Proton Synchrotron (SPS) is used to increase their energy further to 450 GeV before they are at last injected (over a period of several minutes) into the main ring. Here, the proton bunches are accumulated, accelerated (over a period of 20 minutes) to their peak energy, and finally circulated for 5 to 24 hours while collisions occur at the four intersection points.[42]
The LHC physics programme is mainly based on proton–proton collisions. However, during shorter running periods, typically one month per year, heavy-ion collisions are included in the programme. While lighter ions are considered as well, the baseline scheme deals with lead ions[43] (see A Large Ion Collider Experiment). The lead ions are first accelerated by the linear accelerator LINAC 3, and the Low Energy Ion Ring (LEIR) is used as an ion storage and cooler unit. The ions are then further accelerated by the PS and SPS before being injected into LHC ring, where they reach an energy of 2.3 TeV per nucleon (or 522 TeV per ion),[44] higher than the energies reached by the Relativistic Heavy Ion Collider. The aim of the heavy-ion programme is to investigate quark–gluon plasma, which existed in the early universe.[45]
DetectorsSee also: List of Large Hadron Collider experimentsNine detectors have been constructed at the LHC, located underground in large caverns excavated at the LHC's intersection points. Two of them, the ATLAS experiment and the Compact Muon Solenoid (CMS), are large general-purpose particle detectors.[2] ALICE and LHCb have more specialized roles and the other five, TOTEM, MoEDAL, LHCf, SND and FASER, are much smaller and are for very specialized research. The ATLAS and CMS experiments discovered the Higgs boson, which is strong evidence that the Standard Model has the correct mechanism of giving mass to elementary particles.[46]CMS detector for LHCComputing and analysis facilitiesMain article: Worldwide LHC Computing GridData produced by LHC, as well as LHC-related simulation, were estimated at approximately 15 petabytes per year (max throughput while running is not stated)[47]—a major challenge in its own right at the time.
The LHC Computing Grid[48] was constructed as part of the LHC design, to handle the massive amounts of data expected for its collisions. It is an international collaborative project that consists of a grid-based computer network infrastructure initially connecting 140 computing centres in 35 countries (over 170 in 36 countries as of 2012). It was designed by CERN to handle the significant volume of data produced by LHC experiments,[49][50] incorporating both private fibre optic cable links and existing high-speed portions of the public Internet to enable data transfer from CERN to academic institutions around the world.[51] The Open Science Grid is used as the primary infrastructure in the United States, and also as part of an interoperable federation with the LHC Computing Grid.
The distributed computing project LHC@home was started to support the construction and calibration of the LHC. The project uses the BOINC platform, enabling anybody with an Internet connection and a computer running Mac OS X, Windows or Linux to use their computer's idle time to simulate how particles will travel in the beam pipes. With this information, the scientists are able to determine how the magnets should be calibrated to gain the most stable "orbit" of the beams in the ring.[52] In August 2011, a second application (Test4Theory) went live which performs simulations against which to compare actual test data, to determine confidence levels of the results.
By 2012, data from over 6 quadrillion (6×1015) LHC proton–proton collisions had been analysed,[53] LHC collision data was being produced at approximately 25 petabytes per year, and the LHC Computing Grid had become the world's largest computing grid in 2012, comprising over 170 computing facilities in a worldwide network across 36 countries.[54][55][56]
Operational historyThe LHC first went operational on 10 September 2008,[57] but initial testing was delayed for 14 months from 19 September 2008 to 20 November 2009, following a magnet quench incident that caused extensive damage to over 50 superconducting magnets, their mountings, and the vacuum pipe.[58][59][60][61][62]
During its first run (2010–2013), the LHC collided two opposing particle beams of either protons at up to 4 teraelectronvolts (4 TeV or 0.64 microjoules), or lead nuclei (574 TeV per nucleus, or 2.76 TeV per nucleon).[63][64] Its first run discoveries included the long-sought Higgs boson, several composite particles (hadrons) like the χb (3P) bottomonium state, the first creation of a quark–gluon plasma, and the first observations of the very rare decay of the Bs meson into two muons (Bs0 → μ+μ−), which challenged the validity of existing models of challengesThe size of the LHC constitutes an exceptional engineering challenge with unique operational issues on account of the amount of energy stored in the magnets and the beams.[42][66] While operating, the total energy stored in the magnets is 10 GJ (2,400 kilograms of TNT) and the total energy carried by the two beams reaches 724 MJ (173 kilograms of TNT).[67]
Loss of only one ten-millionth part (10−7) of the beam is sufficient to quench a superconducting magnet, while each of the two beam dumps must absorb 362 MJ (87 kilograms of TNT). These energies are carried by very little matter: under nominal operating conditions (2,808 bunches per beam, 1.15×1011 protons per bunch), the beam pipes contain 1.0×10−9 gram of hydrogen, which, in standard conditions for temperature and pressure, would fill the volume of one grain of fine sand.
CostSee also: List of megaprojectsWith a budget of €7.5 billion (approx. $9bn or £6.19bn as of June 2010), the LHC is one of the most expensive scientific instruments[1] ever built.[68] The total cost of the project is expected to be of the order of 4.6bn Swiss francs (SFr) (approx. $4.4bn, €3.1bn, or £2.8bn as of January 2010) for the accelerator and 1.16bn (SFr) (approx. $1.1bn, €0.8bn, or £0.7bn as of January 2010) for the CERN contribution to the experiments.[69]
The construction of LHC was approved in 1995 with a budget of SFr 2.6bn, with another SFr 210M toward the experiments. However, cost overruns, estimated in a major review in 2001 at around SFr 480M for the accelerator, and SFr 50M for the experiments, along with a reduction in CERN's budget, pushed the completion date from 2005 to April 2007.[70] The superconducting magnets were responsible for SFr 180M of the cost increase. There were also further costs and delays owing to engineering difficulties encountered while building the cavern for the Compact Muon Solenoid,[71] and also due to magnet supports which were insufficiently strongly designed and failed their initial testing (2007) and damage from a magnet quench and liquid helium escape (inaugural testing, 2008) (see: Construction accidents and delays).[72] Because electricity costs are lower during the summer, the LHC normally does not operate over the winter months,[73] although exceptions over the 2009/10 and 2012/2013 winters were made to make up for the 2008 start-up delays and to improve precision of measurements of the new particle discovered in 2012, respectively.
Construction accidents and delaysOn 25 October 2005, José Pereira Lages, a technician, was killed in the LHC when a switchgear that was being transported fell on top of him.[74]On 27 March 2007, a cryogenic magnet support designed and provided by Fermilab and KEK broke during an initial pressure test involving one of the LHC's inner triplet (focusing quadrupole) magnet assemblies. No one was injured. Fermilab director Pier Oddone stated "In this case we are dumbfounded that we missed some very simple balance of forces". The fault had been present in the original design, and remained during four engineering reviews over the following years.[75] Analysis revealed that its design, made as thin as possible for better insulation, was not strong enough to withstand the forces generated during pressure testing. Details are available in a statement from Fermilab, with which CERN is in agreement.[76][77] Repairing the broken magnet and reinforcing the eight identical assemblies used by LHC delayed the start-up date, then planned for November 2007.On 19 September 2008, during initial testing, a faulty electrical connection led to a magnet quench (the sudden loss of a superconducting magnet's superconducting ability owing to warming or electric field effects). Six tonnes of supercooled liquid helium—used to cool the magnets—escaped, with sufficient force to break 10-ton magnets nearby from their mountings, and caused considerable damage and contamination of the vacuum tube. Repairs and safety checks caused a delay of around 14 months.[78][79][80]Two vacuum leaks were found in July 2009, and the start of operations was further postponed to mid-November 2009.[81]Exclusion of RussiaWith the 2022 invasion of Ukraine by Russia, the participation of Russians with CERN was called into question. Approximately 8% of the workforce are of Russian nationality. In June 2022 CERN said the governing council "intends to terminate" CERN's cooperation agreements with Belarus and Russia when they expire, respectively in June and December 2024. CERN said it would monitor developments in Ukraine and remains prepared to take additional steps as warranted.[82][83] CERN further said that it would reduce the Ukrainian contribution to CERN for 2022 to the amount already remitted to the Organization, thereby waiving the second instalment of the contribution.[84]
Initial lower magnet currentsMain article: Superconducting magnet § Magnet "training"In both of its runs (2010 to 2012 and 2015), the LHC was initially run at energies below its planned operating energy, and ramped up to just 2 x 4 TeV energy on its first run and 2 x 6.5 TeV on its second run, below the design energy of 2 x 7 TeV. This is because massive superconducting magnets require considerable magnet training to handle the high currents involved without losing their superconducting ability, and the high currents are necessary to allow a high proton energy. The "training" process involves repeatedly running the magnets with lower currents to provoke any quenches or minute movements that may result. It also takes time to cool down magnets to their operating temperature of around 1.9 K (close to absolute zero). Over time the magnet "beds in" and ceases to quench at these lesser currents and can handle the full design current without quenching; CERN media describe the magnets as "shaking out" the unavoidable tiny manufacturing imperfections in their crystals and positions that had initially impaired their ability to handle their planned currents. The magnets, over time and with training, gradually become able to handle their full planned currents without quenching.[85][86]
Inaugural tests (2008)The first beam was circulated through the collider on the morning of 10 September 2008.[87] CERN successfully fired the protons around the tunnel in stages, three kilometres at a time. The particles were fired in a clockwise direction into the accelerator and successfully steered around it at 10:28 local time.[57] The LHC successfully completed its major test: after a series of trial runs, two white dots flashed on a computer screen showing the protons travelled the full length of the collider. It took less than one hour to guide the stream of particles around its inaugural circuit.[88] CERN next successfully sent a beam of protons in an anticlockwise direction, taking slightly longer at one and a half hours owing to a problem with the cryogenics, with the full circuit being completed at 14:59.
Quench incident
Wikinews has related news:CERN says repairs to LHC particle accelerator to cost US$21 millionOn 19 September 2008, a magnet quench occurred in about 100 bending magnets in sectors 3 and 4, where an electrical fault led to a loss of approximately six tonnes of liquid helium (the magnets' cryogenic coolant), which was vented into the tunnel. The escaping vapour expanded with explosive force, damaging a total of 53 superconducting magnets and their mountings, and contaminating the vacuum pipe, which also lost vacuum conditions.[58][59][89]
Shortly after the incident, CERN reported that the most likely cause of the problem was a faulty electrical connection between two magnets, and that – owing to the time needed to warm up the affected sectors and then cool them back down to operating temperature – it would take at least two months to fix.[90] CERN released an interim technical report[89] and preliminary analysis of the incident on 15 and 16 October 2008 respectively,[91] and a more detailed report on 5 December 2008.[79] The analysis of the incident by CERN confirmed that an electrical fault had indeed been the cause. The faulty electrical connection had led (correctly) to a failsafe power abort of the electrical systems powering the superconducting magnets, but had also caused an electric arc (or discharge) which damaged the integrity of the supercooled helium's enclosure and vacuum insulation, causing the coolant's temperature and pressure to rapidly rise beyond the ability of the safety systems to contain it,[89] and leading to a temperature rise of about 100 degrees Celsius in some of the affected magnets. Energy stored in the superconducting magnets and electrical noise induced in other quench detectors also played a role in the rapid heating. Around two tonnes of liquid helium escaped explosively before detectors triggered an emergency stop, and a further four tonnes leaked at lower pressure in the aftermath.[89] A total of 53 magnets were damaged in the incident and were repaired or replaced during the winter shutdown.[92] This accident was thoroughly discussed in a 22 February 2010 Superconductor Science and Technology article by CERN physicist Lucio Rossi.[93]
In the original schedule for LHC commissioning, the first "modest" high-energy collisions at a centre-of-mass energy of 900 GeV were expected to take place before the end of September 2008, and the LHC was expected to be operating at 10 TeV by the end of 2008.[94] However, owing to the delay caused by the incident, the collider was not operational until November 2009.[95] Despite the delay, LHC was officially inaugurated on 21 October 2008, in the presence of political leaders, science ministers from CERN's 20 Member States, CERN officials, and members of the worldwide scientific community.[96]
Most of 2009 was spent on repairs and reviews from the damage caused by the quench incident, along with two further vacuum leaks identified in July 2009; this pushed the start of operations to November of that year.[81]
Run 1: first operational run (2009–2013)
Seminar on the physics of LHC by John Iliopoulos (2009).[97]On 20 November 2009, low-energy beams circulated in the tunnel for the first time since the incident, and shortly after, on 30 November, the LHC achieved 1.18 TeV per beam to become the world's highest-energy particle accelerator, beating the Tevatron's previous record of 0.98 TeV per beam held for eight years.[98]
The early part of 2010 saw the continued ramp-up of beam in energies and early physics experiments towards 3.5 TeV per beam and on 30 March 2010, LHC set a new record for high-energy collisions by colliding proton beams at a combined energy level of 7 TeV. The attempt was the third that day, after two unsuccessful attempts in which the protons had to be "dumped" from the collider and new beams had to be injected.[99] This also marked the start of the main research programme.
The first proton run ended on 4 November 2010. A run with lead ions started on 8 November 2010, and ended on 6 December 2010,[100] allowing the ALICE experiment to study matter under extreme conditions similar to those shortly after the Big Bang.[101]
CERN originally planned that the LHC would run through to the end of 2012, with a short break at the end of 2011 to allow for an increase in beam energy from 3.5 to 4 TeV per beam.[5] At the end of 2012, the LHC was planned to be temporarily shut down until around 2015 to allow upgrade to a planned beam energy of 7 TeV per beam.[102] In late 2012, in light of the July 2012 discovery of the Higgs boson, the shutdown was postponed for some weeks into early 2013, to allow additional data to be obtained before shutdown.
Long Shutdown 1 (2013–2015)
A section of the LHCThe LHC was shut down on 13 February 2013 for its 2-year upgrade called Long Shutdown 1 (LS1), which was to touch on many aspects of the LHC: enabling collisions at 14 TeV, enhancing its detectors and pre-accelerators (the Proton Synchrotron and Super Proton Synchrotron), as well as replacing its ventilation system and 100 km (62 mi) of cabling impaired by high-energy collisions from its first run.[103] The upgraded collider began its long start-up and testing process in June 2014, with the Proton Synchrotron Booster starting on 2 June 2014, the final interconnection between magnets completing and the Proton Synchrotron circulating particles on 18 June 2014, and the first section of the main LHC supermagnet system reaching operating temperature of 1.9 K (−271.25 °C), a few days later.[104] Due to the slow progress with "training" the superconducting magnets, it was decided to start the second run with a lower energy of 6.5 TeV per beam, corresponding to a current of 11,000 amperes. The first of the main LHC magnets were reported to have been successfully trained by 9 December 2014, while training the other magnet sectors was finished in March 2015.[105]
Run 2: second operational run (2015–2018)On 5 April 2015, the LHC restarted after a two-year break, during which the electrical connectors between the bending magnets were upgraded to safely handle the current required for 7 TeV per beam (14 TeV collision energy).[6][106] However, the bending magnets were only trained to handle up to 6.5 TeV per beam (13 TeV collision energy), which became the operating energy for 2015 to 2018.[85] The energy was first reached on 10 April 2015.[107] The upgrades culminated in colliding protons together with a combined energy of 13 TeV.[108] On 3 June 2015, the LHC started delivering physics data after almost two years offline.[109] In the following months, it was used for proton–proton collisions, while in November, the machine switched to collisions of lead ions and in December, the usual winter shutdown started.
In 2016, the machine operators focused on increasing the luminosity for proton–proton collisions. The design value was first reached 29 June,[40] and further improvements increased the collision rate to 40% above the design value.[110] The total number of collisions in 2016 exceeded the number from Run 1 – at a higher energy per collision. The proton–proton run was followed by four weeks of proton–lead collisions.[111]
In 2017, the luminosity was increased further and reached twice the design value. The total number of collisions was higher than in 2016 as well.[41]
The 2018 physics run began on 17 April and stopped on 3 December, including four weeks of lead–lead collisions.[112]
Long Shutdown 2 (2018–2022)Long Shutdown 2 (LS2) started on 10 December 2018. The LHC and the whole CERN accelerator complex was maintained and upgraded. The goal of the upgrades was to implement the High Luminosity Large Hadron Collider (HL-LHC) project that will increase the luminosity by a factor of 10. LS2 ended in April 2022. The Long Shutdown 3 (LS3) in the 2020s will take place before the HL-LHC project is done.
Run 3: third operational run (2022)LHC became operational again on 22 April 2022 with a new maximum beam energy of 6.8 TeV (13.6 TeV collision energy), which was first achieved on 25 April.[113][114] It officially commenced its run 3 physics season on July 5, 2022. [115] This round is expected to continue until 2026.[116] In addition to a higher energy the LHC is expected to reach a higher luminosity, which is expected to increase even further with the upgrade to the HL-LHC after Run 3.[117]
Timeline of operationsBeyond the Standard ModelCMS Higgs-event.jpgSimulated Large Hadron Collider CMS particle detector data depicting a Higgs boson produced by colliding protons decaying into hadron jets and electronsStandard gravityExperimentsANNIEGran Event10 Sep 2008 CERN successfully fired the first protons around the entire tunnel circuit in stages.19 Sep 2008 Magnetic quench occurred in about 100 bending magnets in sectors 3 and 4, causing a loss of approximately 6 tonnes of liquid helium.30 Sep 2008 First "modest" high-energy collisions planned but postponed due to accident.[33]16 Oct 2008 CERN released a preliminary analysis of the accident.21 Oct 2008 Official inauguration.5 Dec 2008 CERN released detailed analysis.20 Nov 2009 Low-energy beams circulated in the tunnel for the first time since the accident.[61]23 Nov 2009 First particle collisions in all four detectors at 450 GeV.30 Nov 2009 LHC becomes the world's highest-energy particle accelerator achieving 1.18 TeV per beam, beating the Tevatron's previous record of 0.98 TeV per beam held for eight years.[118]15 Dec 2009 First scientific results, covering 284 collisions in the ALICE detector.[119]30 Mar 2010 The two beams collided at 7 TeV (3.5 TeV per beam) in the LHC at 13:06 CEST, marking the start of the LHC research programme.8 Nov 2010 Start of the first run with lead ions.6 Dec 2010 End of the run with lead ions. Shutdown until early 2011.13 Mar 2011 Beginning of the 2011 run with proton beams.[120]21 Apr 2011 LHC becomes the world's highest-luminosity hadron accelerator achieving a peak luminosity of 4.67·1032 cm−2s−1, beating the Tevatron's previous record of 4·1032 cm−2s−1 held for one year.[121]24 May 2011 ALICE reports that a Quark–gluon plasma has been achieved with earlier lead collisions.[122]17 Jun 2011 The high-luminosity experiments ATLAS and CMS reach 1 fb−1 of collected data.[123]14 Oct 2011 LHCb reaches 1 fb−1 of collected data.[124]23 Oct 2011 The high-luminosity experiments ATLAS and CMS reach 5 fb−1 of collected data.Nov 2011 Second run with lead ions.22 Dec 2011 First new composite particle discovery, the χb (3P) bottomonium meson, observed with proton–proton collisions in 2011.[125]5 Apr 2012 First collisions with stable beams in 2012 after the winter shutdown. The energy is increased to 4 TeV per beam (8 TeV in collisions).[126]4 Jul 2012 First new elementary particle discovery, a new boson observed that is "consistent with" the theorized Higgs boson. (This has now been confirmed as the Higgs boson itself.[127])8 Nov 2012 First observation of the very rare decay of the Bs meson into two muons (Bs0 → μ+μ−), a major test of supersymmetry theories,[128] shows results at 3.5 sigma that match the Standard Model rather than many of its super-symmetrical variants.20 Jan 2013 Start of the first run colliding protons with lead ions.11 Feb 2013 End of the first run colliding protons with lead ions.14 Feb 2013 Beginning of the first long shutdown to prepare the collider for a higher energy and luminosity.[129]Long Shutdown 17 Mar 2015 Injection tests for Run 2 send protons towards LHCb & ALICE5 Apr 2015 Both beams circulated in the collider.[6] Four days later, a new record energy of 6.5 TeV per proton was achieved.[130]20 May 2015 Protons collided in the LHC at the record-breaking collision energy of 13 TeV.[108]3 Jun 2015 Start of delivering the physics data after almost two years offline for recommissioning.[109]4 Nov 2015 End of proton collisions in 2015, start of preparations for ion collisions.Nov 2015 Ion collisions at a record-breaking energy of more than 1 PeV (1015 eV)[131]13 Dec 2015 End of ion collisions in 201523 Apr 2016 Data-taking in 2016 begins29 June 2016 The LHC achieves a luminosity of 1.0 · 1034 cm−2s−1, its design value.[40] Further improvements over the year increased the luminosity to 40% above the design value.[110]26 Oct 2016 End of 2016 proton–proton collisions10 Nov 2016 Beginning of 2016 proton–lead collisions3 Dec 2016 End of 2016 proton–lead collisions24 May 2017 Start of 2017 proton–proton collisions. During 2017, the luminosity increased to twice its design value.[41]10 Nov 2017 End of regular 2017 proton–proton collision mode.[41]17 Apr 2018 Start of 2018 proton–proton collisions.12 Nov 2018 End of 2018 proton operations at CERN.[132]3 Dec 2018 End of 2018 lead-ion run.[132]10 Dec 2018 End of 2018 physics operation and start of Long Shutdown 2.[132]Long Shutdown 222 Apr 2022 LHC becomes operational again.[133]Findings and discoveriesAn initial focus of research was to investigate the possible existence of the Higgs boson, a key part of the Standard Model of physics which was predicted by theory, but had not yet been observed before due to its high mass and elusive nature. CERN scientists estimated that, if the Standard Model was correct, the LHC would produce several Higgs bosons every minute, allowing physicists to finally confirm or disprove the Higgs boson's existence. In addition, the LHC allowed the search for supersymmetric particles and other hypothetical particles as possible unknown areas of physics.[63] Some extensions of the Standard Model predict additional particles, such as the heavy W' and Z' gauge bosons, which are also estimated to be within reach of the LHC to discover.[134]
First run (data taken 2009–2013)The first physics results from the LHC, involving 284 collisions which took place in the ALICE detector, were reported on 15 December 2009.[119] The results of the first proton–proton collisions at energies higher than Fermilab's Tevatron proton–antiproton collisions were published by the CMS collaboration in early February 2010, yielding greater-than-predicted charged-hadron production.[135]
After the first year of data collection, the LHC experimental collaborations started to release their preliminary results concerning searches for new physics beyond the Standard Model in proton–proton collisions.[136][137][138][139] No evidence of new particles was detected in the 2010 data. As a result, bounds were set on the allowed parameter space of various extensions of the Standard Model, such as models with large extra dimensions, constrained versions of the Minimal Supersymmetric Standard Model, and others.[140][141][142]
On 24 May 2011, it was reported that quark–gluon plasma (the densest matter thought to exist besides black holes) had been created in the LHC.[122]A Feynman diagram of one way the Higgs boson may be produced at the LHC. Here, two quarks each emit a W or Z boson, which combine to make a neutral Higgs.Between July and August 2011, results of searches for the Higgs boson and for exotic particles, based on the data collected during the first half of the 2011 run, were presented in conferences in Grenoble[143] and Mumbai.[144] In the latter conference, it was reported that, despite hints of a Higgs signal in earlier data, ATLAS and CMS exclude with 95% confidence level (using the CLs method) the existence of a Higgs boson with the properties predicted by the Standard Model over most of the mass region between 145 and 466 GeV.[145] The searches for new particles did not yield signals either, allowing to further constrain the parameter space of various extensions of the Standard Model, including its supersymmetric extensions.[146][147]
On 13 December 2011, CERN reported that the Standard Model Higgs boson, if it exists, is most likely to have a mass constrained to the range 115–130 GeV. Both the CMS and ATLAS detectors have also shown intensity peaks in the 124–125 GeV range, consistent with either background noise or the observation of the Higgs boson.[148]
On 22 December 2011, it was reported that a new composite particle had been observed, the χb (3P) bottomonium state.[125]
On 4 July 2012, both the CMS and ATLAS teams announced the discovery of a boson in the mass region around 125–126 GeV, with a statistical significance at the level of 5 sigma each. This meets the formal level required to announce a new particle. The observed properties were consistent with the Higgs boson, but scientists were cautious as to whether it is formally identified as actually being the Higgs boson, pending further analysis.[149] On 14 March 2013, CERN announced confirmation that the observed particle was indeed the predicted Higgs boson.[150]
On 8 November 2012, the LHCb team reported on an experiment seen as a "golden" test of supersymmetry theories in physics,[128] by measuring the very rare decay of theB_{s} meson into two muons (0→+−{\displaystyle B_{s}^{0}ightarrow \mu ^{+}\mu ^{-}}). The results, which match those predicted by the non-supersymmetrical Standard Model rather than the predictions of many branches of supersymmetry, show the decays are less common than some forms of supersymmetry predict, though could still match the predictions of other versions of supersymmetry theory. The results as initially drafted are stated to be short of proof but at a relatively high 3.5 sigma level of significance.[151] The result was later confirmed by the CMS collaboration.[152]
In August 2013, the LHCb team revealed an anomaly in the angular distribution of B meson decay products which could not be predicted by the Standard Model; this anomaly had a statistical certainty of 4.5 sigma, just short of the 5 sigma needed to be officially recognized as a discovery. It is unknown what the cause of this anomaly would be, although the Z' boson has been suggested as a possible candidate.[153]
On 19 November 2014, the LHCb experiment announced the discovery of two new heavy subatomic particles,Ξ′−b andΞ∗−b. Both of them are baryons that are composed of one bottom, one down, and one strange quark. They are excited states of the bottom Xi baryon.[154][155]
The LHCb collaboration has observed multiple exotic hadrons, possibly pentaquarks or tetraquarks, in the Run 1 data. On 4 April 2014, the collaboration confirmed the existence of the tetraquark candidate Z(4430) with a significance of over 13.9 sigma.[156][157] On 13 July 2015, results consistent with pentaquark states in the decay of bottom Lambda baryons (Λ0b) were reported.[158][159][160]
On 28 June 2016, the collaboration announced four tetraquark-like particles decaying into a J/ψ and a φ meson, only one of which was well established before (X(4274), X(4500) and X(4700) and X(4140)).[161][162]
In December 2016, ATLAS presented a measurement of the W boson mass, researching the precision of analyses done at the Tevatron.[163]
Second run (2015–2018)At the conference EPS-HEP 2015 in July, the collaborations presented first cross-section measurements of several particles at the higher collision energy.
On 15 December 2015, the ATLAS and CMS experiments both reported a number of preliminary results for Higgs physics, supersymmetry (SUSY) searches and exotics searches using 13 TeV proton collision data. Both experiments saw a moderate excess around 750 GeV in the two-photon invariant mass spectrum,[164][165][166] but the experiments did not confirm the existence of the hypothetical particle in an August 2016 report.[167][168][169]
In July 2017, many analyses based on the large dataset collected in 2016 were shown. The properties of the Higgs boson were studied in more detail and the precision of many other results was improved.[170]
As of March 2021, the LHC experiments have discovered 59 new hadrons in the data collected during the first two runs.[171]
On 5 July 2022 LHCb reported the discovery of a new type of pentaquark made up of a charm quark and a charm antiquark and an up, a down and a strange quark, observed in an analysis of decays of charged B mesons.[172]
Planned "high-luminosity" upgradeMain article: High Luminosity Large Hadron ColliderAfter some years of running, any particle physics experiment typically begins to suffer from diminishing returns: as the key results reachable by the device begin to be completed, later years of operation discover proportionately less than earlier years. A common response is to upgrade the devices involved, typically in collision energy, luminosity, or improved detectors. In addition to a possible increase to 14 TeV collision energy, a luminosity upgrade of the LHC, called the High Luminosity Large Hadron Collider, started in June 2018 that will boost the accelerator's potential for new discoveries in physics, starting in 2027.[173] The upgrade aims at increasing the luminosity of the machine by a factor of 10, up to 1035 cm−2s−1, providing a better chance to see rare processes and improving statistically marginal measurements.[117]
Planned Future Circular Collider (FCC)CERN has several preliminary designs for a Future Circular Collider (FCC) — which would be the most powerful particle smasher ever built — with different types of collider ranging in cost from around €9 billion (US$10.2 billion) to €21 billion. It is CERN’s opening offer in a priority-setting process called the European Strategy for Particle Physics Update, and will affect the field’s future well into the second half of the century. Not everyone is convinced that the FCC is a good investment. “There is no reason to think that there should be new physics in the energy regime that such a collider would reach,” says Sabine Hossenfelder, a theoretical physicist at the Frankfurt Institute for Advanced Studies in Germany. Hossenfelder says that the large sums involved might be better spent on other types of huge facility. For example, she says that placing a major radio telescope on the far side of the Moon, or a gravitational-wave detector in orbit, would both be safer bets than the collider in terms of their return on science.[174]
Safety of particle collisionsMain article: Safety of high-energy particle collision experimentsThe experiments at the Large Hadron Collider sparked fears that the particle collisions might produce doomsday phenomena, involving the production of stable microscopic black holes or the creation of hypothetical particles called strangelets.[175] Two CERN-commissioned safety reviews examined these concerns and concluded that the experiments at the LHC present no danger and that there is no reason for concern,[176][177][178] a conclusion endorsed by the American Physical Society.[179]
The reports also noted that the physical conditions and collision events that exist in the LHC and similar experiments occur naturally and routinely in the universe without hazardous consequences,[177] including ultra-high-energy cosmic rays observed to impact Earth with energies far higher than those in any human-made collider.
Popular cultureThe Large Hadron Collider gained a considerable amount of attention from outside the scientific community and its progress is followed by most popular science media. The LHC has also inspired works of fiction including novels, TV series, video games and films.
CERN employee Katherine McAlpine's "Large Hadron Rap"[180] surpassed 8 million YouTube views as of 2022.[181][182]
The band Les Horribles Cernettes was founded by women from CERN. The name was chosen so to have the same initials as the LHC.[183][184]
National Geographic Channel's World's Toughest Fixes, Season 2 (2010), Episode 6 "Atom Smasher" features the replacement of the last superconducting magnet section in the repair of the collider after the 2008 quench incident. The episode includes actual footage from the repair facility to the inside of the collider, and explanations of the function, engineering, and purpose of the LHC.[185]
The song "Munich" off of the 2012 studio album Scars & Stories by The Fray is inspired by the LHC. Lead singer Isaac Slade said in an interview with The Huffington Post, "There's this large particle collider out in Switzerland that is kind of helping scientists peel back the curtain on what creates gravity and mass. Some very big questions are being raised, even some things that Einstein proposed, that have just been accepted for decades are starting to be challenged. They're looking for the God Particle, basically, the particle that holds it all together. That song is really just about the mystery of why we're all here and what's holding it all together, you know?" [186]
The Large Hadron Collider was the focus of the 2012 student film Decay, with the movie being filmed on location in CERN's maintenance tunnels.[187]
FictionThe novel Angels & Demons, by Dan Brown, involves antimatter created at the LHC to be used in a weapon against the Vatican. In response, CERN published a "Fact or Fiction?" page discussing the accuracy of the book's portrayal of the LHC, CERN, and particle physics in general.[188] The movie version of the book has footage filmed on-site at one of the experiments at the LHC; the director, Ron Howard, met with CERN experts in an effort to make the science in the story more accurate.[189]
The novel FlashForward, by Robert J. Sawyer, involves the search for the Higgs boson at the LHC. CERN published a "Science and Fiction" page interviewing Sawyer and physicists about the book and the TV series based on it.[190]
In the American sitcom The Big Bang Theory episode "The Large Hadron Collision" (season 3 episode 15), Leonard is offered a chance to visit the Large
In particle physics, a hadron (/ˈhædrɒn/ (listen); Ancient Greek: ἁδρός, romanized: hadrós; "stout, thick") is a composite subatomic particle made of two or more quarks held together by the strong interaction. They are analogous to molecules that are held together by the electric force. Most of the mass of ordinary matter comes from two hadrons: the proton and the neutron, while most of the mass of the protons and neutrons is in turn due to the binding energy of their constituent quarks, due to the strong force.
Hadrons are categorized into two broad families: baryons, made of an odd number of quarks (usually three quarks) and mesons, made of an even number of quarks (usually two quarks: one quark and one antiquark).[1] Protons and neutrons (which make the majority of the mass of an atom) are examples of baryons; pions are an example of a meson. "Exotic" hadrons, containing more than three valence quarks, have been discovered in recent years. A tetraquark state (an exotic meson), named the Z(4430)−, was discovered in 2007 by the Belle Collaboration[2] and confirmed as a resonance in 2014 by the LHCb collaboration.[3] Two pentaquark states (exotic baryons), named P+c(4380) and P+c(4450), were discovered in 2015 by the LHCb collaboration.[4] There are several more exotic hadron candidates and other colour-singlet quark combinations that may also exist.
Almost all "free" hadrons and antihadrons (meaning, in isolation and not bound within an atomic nucleus) are believed to be unstable and eventually decay into other particles. The only known possible exception is free protons, which appear to be stable, or at least, take immense amounts of time to decay (order of 1034+ years). By way of comparison, free neutrons are the longest-lived unstable particle, and decay with a half-life of about 879 seconds.[a][5] Experimentally, hadron physics is studied by colliding hadrons, e.g. protons, with each other or the nuclei of dense, heavy elements, such as lead or gold, and detecting the debris in the produced particle showers. A similar process occurs in the natural environment, in the extreme upper-atmosphere, where muons and mesons such as pions are produced by the collisions of cosmic rays with rarefied gas particles in the outer atmosphere.[6]
Terminology and etymologyThe term "hadron" is a new Greek word introduced by L.B. Okun and in a plenary talk at the 1962 International Conference on High Energy Physics at CERN.[7] He opened his talk with the definition of a new category term:
Notwithstanding the fact that this report deals with weak interactions, we shall frequently have to speak of strongly interacting particles. These particles pose not only numerous scientific problems, but also a terminological problem. The point is that "strongly interacting particles" is a very clumsy term which does not yield itself to the formation of an adjective. For this reason, to take but one instance, decays into strongly interacting particles are called "non-leptonic". This definition is not exact because "non-leptonic" may also signify photonic. In this report I shall call strongly interacting particles "hadrons", and the corresponding decays "hadronic" (the Greek ἁδρός signifies "large", "massive", in contrast to λεπτός which means "small", "light"). I hope that this terminology will prove to be convenient. — L.B. Okun (1962)[7]
PropertiesA green and a magenta ("antigreen") arrow canceling out each other out white, representing a meson; a red, a green, and a blue arrow canceling out to white, representing a baryon; a yellow ("antiblue"), a magenta, and a cyan ("antired") arrow canceling out to white, representing an antibaryon.All types of hadrons have zero total color charge (three examples shown)According to the quark model,[8] the properties of hadrons are primarily determined by their so-called valence quarks. For example, a proton is composed of two up quarks (each with electric charge ++2⁄3, for a total of +4⁄3 together) and one down quark (with electric charge −+1⁄3). Adding these together yields the proton charge of +1. Although quarks also carry color charge, hadrons must have zero total color charge because of a phenomenon called color confinement. That is, hadrons must be "colorless" or "white". The simplest ways for this to occur are with a quark of one color and an antiquark of the corresponding anticolor, or three quarks of different colors. Hadrons with the first arrangement are a type of meson, and those with the second arrangement are a type of baryon.
Massless virtual gluons compose the overwhelming majority of particles inside hadrons, as well as the major constituents of its mass (with the exception of the heavy charm and bottom quarks; the top quark vanishes before it has time to bind into a hadron). The strength of the strong force gluons which bind the quarks together has sufficient energy (E) to have resonances composed of massive (m) quarks (E ≥ mc2). One outcome is that short-lived pairs of virtual quarks and antiquarks are continually forming and vanishing again inside a hadron. Because the virtual quarks are not stable wave packets (quanta), but an irregular and transient phenomenon, it is not meaningful to ask which quark is real and which virtual; only the small excess is apparent from the outside in the form of a hadron. Therefore, when a hadron or anti-hadron is stated to consist of (typically) 2 or 3 quarks, this technically refers to the constant excess of quarks vs. antiquarks.
Like all subatomic particles, hadrons are assigned quantum numbers corresponding to the representations of the Poincaré group: JPC (m), where J is the spin quantum number, P the intrinsic parity (or P-parity), C the charge conjugation (or C-parity), and m is the particle's mass. Note that the mass of a hadron has very little to do with the mass of its valence quarks; rather, due to mass–energy equivalence, most of the mass comes from the large amount of energy associated with the strong interaction. Hadrons may also carry flavor quantum numbers such as isospin (G parity), and strangeness. All quarks carry an additive, conserved quantum number called a baryon number (B), which is ++1⁄3 for quarks and −+1⁄3 for antiquarks. This means that baryons (composite particles made of three, five or a larger odd number of quarks) have B = 1 whereas mesons have B = 0.
Hadrons have excited states known as resonances. Each ground state hadron may have several excited states; several hundreds of resonances have been observed in experiments. Resonances decay extremely quickly (within about 10−24 seconds) via the strong nuclear force.
In other phases of matter the hadrons may disappear. For example, at very high temperature and high pressure, unless there are sufficiently many flavors of quarks, the theory of quantum chromodynamics (QCD) predicts that quarks and gluons will no longer be confined within hadrons, "because the strength of the strong interaction diminishes with energy". This property, which is known as asymptotic freedom, has been experimentally confirmed in the energy range between 1 GeV (gigaelectronvolt) and 1 TeV (teraelectronvolt).[9] All free hadrons except (possibly) the proton and antiproton are unstable.
BaryonsMain articles: Baryon and Exotic baryonBaryons are hadrons containing an odd number of valence quarks (at least 3).[1] Most well known baryons such as the proton and neutron have three valence quarks, but pentaquarks with five quarks – three quarks of different colors, and also one extra quark-antiquark pair – have also been proven to exist. Because baryons have an odd number of quarks, they are also all fermions, i.e., they have half-integer spin. As quarks possess baryon number B = 1⁄3, baryons have baryon number B = 1. Pentaquarks also have B = 1, since the extra quark's and antiquark's baryon numbers cancel.
Each type of baryon has a corresponding antiparticle (antibaryon) in which quarks are replaced by their corresponding antiquarks. For example, just as a proton is made of two up-quarks and one down-quark, its corresponding antiparticle, the antiproton, is made of two up-antiquarks and one down-antiquark.
As of August 2015, there are two known pentaquarks, P+c(4380) and P+c(4450), both discovered in 2015 by the LHCb collaboration.[4]
MesonsMain articles: Meson and Exotic mesonMesons are hadrons containing an even number of valence quarks (at least 2).[1] Most well known mesons are composed of a quark-antiquark pair, but possible tetraquarks (4 quarks) and hexaquarks (6 quarks, comprising either a dibaryon or three quark-antiquark pairs) may have been discovered and are being investigated to confirm their nature.[10] Several other hypothetical types of exotic meson may exist which do not fall within the quark model of classification. These include glueballs and hybrid mesons (mesons bound by excited gluons).
Because mesons have an even number of quarks, they are also all bosons, with integer spin, i.e., 0, +1, or −1. They have baryon number B =1/3−1/3= 0 . Examples of mesons commonly produced in particle physics experiments include pions and kaons. Pions also play a role in holding atomic nuclei together via the residual strong force.
See alsoExotic hadronHadron therapy, a.k.a. particle therapyHadronization, the formation of hadrons out of quarks and gluonsLarge Hadron Collider (LHC)List of particlesStandard modelSubatomic particlesFootnotesThe proton and neutrons' respective antiparticles are expected to follow the same pattern, but they are difficult to capture and study, because they immediately annihilate on contact with ordinary matter.
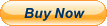
Related Items:
Jack Steinberger- Signed Thank You Card (Nobel Prize 1988)
$48.00
JACK STEINBERGER PHYSICIST NOBEL PRIZE WINNER HADRON NEUTRINO MANUSCRIPT QUARKS
$16365.78
"Nobel Prize Physics" Jack Steinberger Signed 4X5.75 Color Photo COA
$104.99